Source: Glass Structures & Engineering
Authors: Alessandra Luna-Navarro, Eleonora Brembilla, Pedro de la Barra, Louis Moreau & Mauro Overend
DOI: https://doi.org/10.1007/s40940-024-00281-0
Abstract
Visual defects, in particular haze, in glass and façade technologies can significantly impact the aesthetic quality and human experience of daylight and views in buildings. The glass and façade industry increasingly requires methods that can objectively predict and measure the subjective user experience of haze. This is required to appropriately inform the manufacturing process, ensuring optimal functionality and performance, and avoiding material waste and economic losses due to the replacement of defective glazing. Existing methods for measuring haze are not appropriate for assessing large samples, either at manufacturing sites or in-situ. However, haze defects are often only exhibited and visible when glazing is produced in large samples or installed under real luminous conditions. This paper introduces a novel luminance-based method that measures haze by evaluating the halo around a light source that is observed through the glazing.
This method is initially tested in a controlled laboratory setting on small glazing samples with varying level of haze. The halo serves as a proxy for haze severity and it is quantified by using luminance-based measurements. In this work, the newly-proposed method is verified by comparing the ranking in haze severity of different samples as performed by means of a standard haze meter and the newly proposed method. Additionally, the paper examines the dependence of this ranking on factors such as camera setup distance and light intensity. It was found that the proposed method was able to effectively ranks samples differing in haze intensity by more than 0.1 orders of magnitude. Positioning the camera closer to the glazing and using higher light intensity yielded more accurate results. However, for haze levels below 1% and differences smaller than 0.1 orders of magnitude, the accuracy is insufficient. To define the expected level of accuracy of methods for haze characterisation in-situ, the sensitivity of the human eye to haze under varying luminous environments and view content needs to be quantified.
1 Introduction
A few novel high-performing and complex glazing solutions have exhibited visual defects affecting view clarity, or in other words “how clearly the content appears in the window view” (Ko et al. 2022). Defects in glazing can have a detrimental impact on view clarity and users in buildings since they reduce access to outdoor view. The mismatch in expected view clarity performance and actual one is typically due to limited knowledge of the long-term performance of newly-proposed materials, glazing assemblies, and components under real weather conditions or/and when produced in large samples for in-situ installation. For instance, as shown in Fig. 1, this has been the case in novel closed cavity façades, where fogginess has been observed due to poor performance of shading components, while excessive scattering has occurred because of new types of interlayers or recently developed responsive coatings. Haze is currently used in the glass and acrylic industry to describe the visual nuisance perceived by the human eye when looking through a glazing and perceiving excessive light scattering (ASTM D1003-21 Standard Test Method for Haze and Luminous Transmittance of Transparent Plastics 2021).
One of the main barriers to effective production and installation of novel glazing technologies is the lack of methods that can accurately quantify and predict the level of visual nuisance perceived by users when large glazing samples are produced at manufacturing sites or installed in real buildings. The absence of standardized methods for measuring and predicting the human experience with glazing, particularly in terms of view clarity, hinders the manufacturing process and leads to material waste and economic losses, as several façade bays often need to be replaced if the visual defect level is deemed unacceptable. Moreover, there is a significant debate and lack of consensus on what constitutes an acceptable level of visual defects affecting view clarity (Dougie 2024). Acceptance is personal and subjective, while several contextual factors influence individual threshold of acceptance. There are currently not established threshold of acceptance, but industry relies on their own ones, defined case by case. Without an appropriate method to relate objectively measured parameters to the subjective perception of visual nuisance, it is problematic to establish thresholds of acceptability among manufacturers, installers, and building owners.
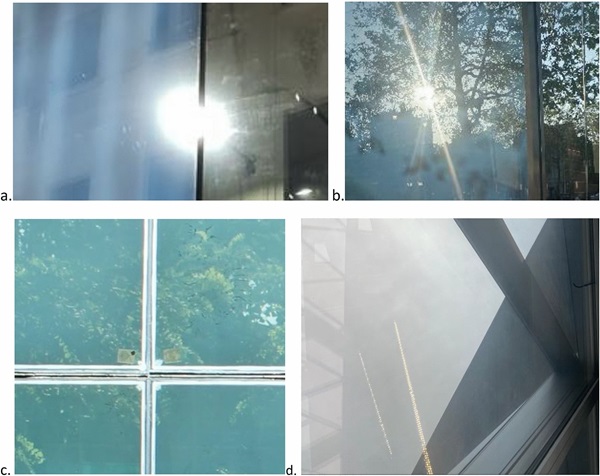
The existing standards ASTM D1003-21 (ASTM D1003-21 Standard Test Method for Haze and Luminous Transmittance of Transparent Plastics 2021), the ISO 14782:2021 (ISO 2021), and the JIS K 7136 (JSA—JIS K 7136 Plastics—Determination of Haze for Transparent Materials 2000), describe measurement procedures for quantifying the level of haze for transparent plastics, but none of the standards describe measurement procedures for glass components. The ASTM D1003-21 defines haze in transmission, as “the scattering of light by a specimen responsible for the reduction in contrast of objects viewed through it. The percent of transmitted light that is scattered so that its direction deviates more than a specified angle from the direction of the incident beam”. In particular, the ISO 14782:2021 defines haze as “an optical property resulting from wide-angle scattering of light”. When light is scattered through a glazing, it can be scattered at wide angle (diffuse scattering), at narrow angles (forward scattering) and backwards (backwards scattering). Depending on the angle of scattering, the visual nuisance perceived can be different.
Both the ASTM D1003-21 and the ISO 14782:2021 standards measure haze by using an apparatus commonly called “haze meter”, which consists of a stabilized light source, an associated optical system, an integrating sphere with ports, and a photometer comprising a photodetector, signal processor and display unit or recorder. In both standards, the level of haze is calculated under a collimated light (i.e. a light source with parallel rays) source impinging on the sample and an integrating sphere to differentiate specular and diffuse light transmission. Haze is then measured as the ratio between the diffuse light transmittance and the total transmitted light (accounting for both direct and diffuse transmittance). While this apparatus can be valid for accurate lab measurements of small specimens, this setup is not ideal for in-situ measurements because of the weight and geometrical constraints, in particular only samples up to ≈5 cm2 can be measured and when glazing build-ups exceed 2–3 cm, the error generated by light scattered outside the integrated sphere is also conspicuous since it can underestimate both the direct-diffuse and direct-hemispherical values. In addition, the the economic value (≈ 20.000 $) of the commercially available apparatus undermines a wide adoption in site. When measured in real building applications, the level of haze is also induced by potential aging or soiling of the installed glazing, therefore it is important to develop methodologies that are applicable in field studies.
Another limitation of the current haze meters is that they can only evaluate scattering under perpendicular collimated sources, while the fraction of light scattered through the glazings may depend on the angle of incidence of the light. In addition, the haze level measured with these methods is dependent on the distance of the sample from the light source. More importantly, current haze meter metrics can be questioned in terms of its validity to truly capture the nuisance of visual perception experienced by the observer (Busato and Perevedentsev 2018), especially since they do not consider the context in which the sample is places. For instance, materials with nominally equal haze values can exhibit substantially different angular distributions of scattered light, which naturally correspond to noticeable differences in transparency (Marasco and Task 2001).
Few alternatives to the haze meters can be found in literature. Busato et al. (2018) recently proposed an image-based method to measure haze, comparing contrast and resolution of the image of a grid viewed through a given sample against the reference image of the bare grid. The authors also found good correlation between their method and haze measurements as per ASTM D-3001; they reported to be able to measure the effect of the airgap distance. However, this method is also made for lab measurements and for small samples. Even if an alternative, simpler low-cost method has been proposed by the same authors (Busato et al. 2021), this was also thought for laboratory measurements of small samples, hence it is not applicable to large glazed surfaces in situ. Marasco and Task (Marasco and Task 2001) tried to relate visual acuity of standard Landolt C targets through haze samples with a method that could be applied to large surfaces but untested for small haze effects typical of architectural facades.
While the previous studies focused on plastics, literature on haze visual effects in glass concerned mainly soiling and dust deposition or visual performance characterization of novel glazing components, such as aerogel glazing or novel coatings. All these studies defined haze as the ratio between diffuse and total transmittance, measured on small samples in laboratory settings by using a spectrophotometer. For instance, Jonsson et al. (2010) measured haze due to dip coated anti-reflection coatings on window glass and electrochromic foil by comparing the diffuse transmittance of the samples with and without coating, measured by using a lab spectrophotometer. The diffuse transmittance was measured by letting the direct transmittance exit the sphere into a beam dump. Buratti et al. (2021) used a similar method to measure the haze effect of novel aerogel systems and considered a haze between 0.01 and 0.09 adequate since close to the one exhibited by standard glazing systems (e.g. 0.01 for float glass). Du et al. (2021) also used a spectrophotometer to measure the haze effect due to air pollution and the impact of daylight availability in an open-space office. Chabas et al. (2008) used the same method for measuring the behaviour of self-cleaning glass. Only one study mentioned haze as a possible defect of laminated glass, whose samples were analysed in a laboratory with a benchtop spectrophotometer (Maduru and Shaik 2022).
Lastly, none of the methods proposed in the literature allow for testing specimens under real daylight conditions and actual view content. Depending on the type of visual defect that is causing the haze, the subjective nuisance perceived by the human eye can vary depending on the angle of incidence of solar radiation, indoor lighting conditions, and the type of view content. For instance, view content with sharp contrasts and geometric patterns may exacerbate perceived visual nuisance, whereas natural views may not. Similarly, poor view content may render visual defects unnoticed by participants, as users in buildings may simply not pay attention or care about the outdoor view. When assessing human-glazing interaction, it is crucial to consider the contextual aspects that influence human satisfaction with glass and façade technologies (Luna-Navarro et al. 2020).
The present study is therefore driven by two main objectives: (i) to overcome current limitations of existing methods regarding the maximum dimension of glass specimens and costs; (ii) to provide new quantitative methods that can better represent and describe the subjective nuisance perceived by users. The goal is to develop a new method based on luminance measured by means of high dynamic range (HDR) photography that can be easily used on large glazing samples, whether at manufacturing sites or in the field once installed, and that can be easily calibrated with subjective nuisance perceived by the human eye, depending on the view content and the light and daylight conditions.
To achieve this, a novel luminance-based methodology is proposed that uses the geometric dispersion and intensity of the halo around a collimated light as a proxy for haze intensity. This halo is produced when light is scattered through glazing. The aim of this paper is to: (1) perform a preliminary evaluation of the potential of the proposed method to capture haze defect by assessing the robustness of the methods in ranking small samples with increasing level of haze in a controlled laboratory environment; (2) evaluate the impact of light intensity and camera distance in measuring the level of haze in comparison to a calibrated haze meter in small samples and controlled laboratory environments.
Section 2 describes the new methodology and the procedure used for testing it. Sections 3 and 4 present and discuss the preliminary results, respectively, which demonstrate the potential of the proposed method to capture haze in comparison to established haze meter devices.
2 Methodology
The novel method devised to detect haze revolves around the identification and measurement of the halo forming around light that shines onto the glass facade, due to the presence of hazy glass. A halo is produced around a light source because of the scattering of light through the medium between the light source and the observer. This phenomenon can be noticeable by a human observer and could therefore be used as a proxy to correlate the haze effect with the subjective perceived level of nuisance (see Fig. 2). To test this assumption an ad-hoc experimental setup was designed and a photography technique to characterise lighting fields was implemented in it.
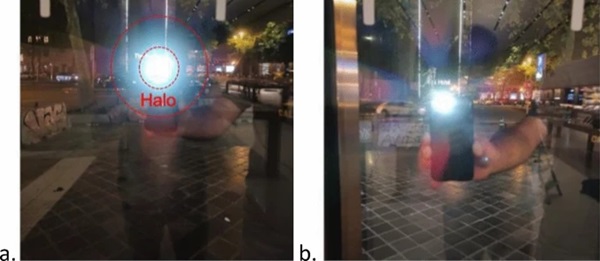
2.1 Experimental setup
The experimental setup was designed to perform HDRI-based luminance measurements on a series of samples with known haze under controlled and repeatable conditions. A series of preliminary experiments informed the setup design in regard to the optimal source of light and view angle of the camera: the source of light had to be large enough to cast a recognisable light patch on the glass while offering the possibility to be collimated; the camera had to be placed at an angle from the light source (i.e. not facing it directly) to avoid any potential lens glare effect and multiple reflections between the camera lens system and the sample. A set of controlled samples with progressive level of haze were produced for the tests, as shown in Fig. 3. These samples were characterised by a relatively low level of haze, ranging from 0.43 to 1.06% as measured by means of standard BYK haze meter. For comparisons, three calibrate BYK haze samples were also used (see Fig. 4). These samples were made of plastic and with a larger haze defect of 1.14%, 4.20% and 10.5% respectively, as measured by means of BYK haze meter. The setup used for the analysis presented in this paper is shown in Fig. 5 and the characteristics of its main components are listed in Table 1.
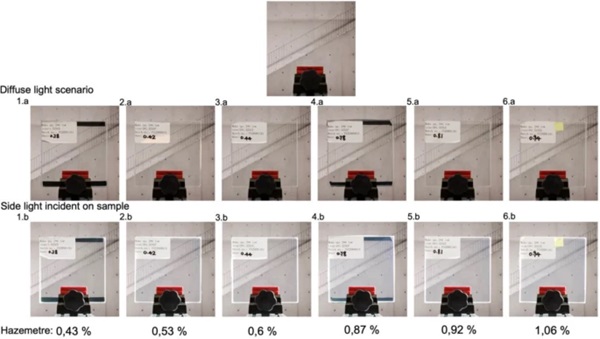
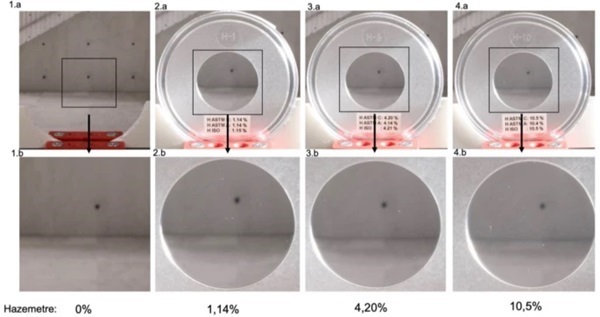
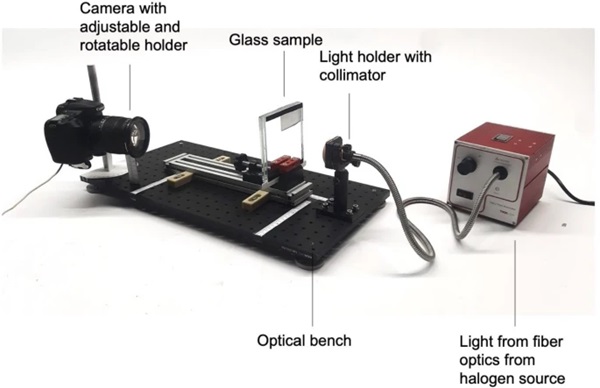
Table 1 Technical specifications of the experimental setup components - Full size table
A collimated light was selected instead of a non-collimated one, to reduce the variability of the results depending on the distance of the light from the glazing. A collimated light enables to perform measurements that are independent from the distance between the source of light and the glazing, but also to produce a halo that is only induced by the haze defect rather than the geometrical light distribution of the source. A laser point source was also considered at the beginning, but then excluded after preliminary tests since the halo induced by the laser beam was not very noticeable, while it was more difficult to control the reflections through the samples and the camera lens system (Table 2).
Table 2 List of samples with known severity of haze used in the experiment - Full size table
One of the objectives of the present work was to determine the best configuration of the experimental setup in terms of light-sample distance and in terms of light source intensity. The combinations tested here are reported in Table 3. These combinations were decided to assess the impact of light intensity and relative distance of the camera to the glazing on the accuracy of the proposed method.
Table 3 Experimental setup configuration tested for this work and associated diagram - Full size table
2.2 HDRI to characterise haze
High Dynamic Range Imaging (HDRI) is a photography technique that allows the capture of a larger exposure range than normal, low-dynamic images. This larger range is comparable to that experienced by the human eye and it therefore allows the photometric characterisation of a scene, i.e. the measurement of luminance values (in cd/m2) from any area visible in the image. The technique consists in collating together a series of images with known, sequential exposure stops and with a known response function relating the range in pixel brightness with the range in relative luminance. The reported error in HDR-based luminance measurements, for well calibrated equipment, is around 10% (Pierson et al. 2021).
In this work, HDRI was used as a mean to compare glass samples with known haze values, therefore testing the capacity of this method to identify and rank haze in laboratory settings. As a preliminary tests, only relative values were taken into account but we plan to carry out absolute calibration and measurements in future tests. In the analysis presented here, the focus was on determining the minimum haze level that is detected by HDRI, considering the characteristic error of this technique, and on comparing the ranking of measured luminance values against the given ranking of the available samples. The camera settings used during the HDRI capture process are reported in Table 4. The white balance was set to daylight, but in future studies the white balance will be set on the artificial light source specifics to improve accuracy.
Table 4 Camera settings for the HDR imaging process - Full size table
The images collected via the HDRI technique were post-processed using Radiance commands and python scripts. The complete workflow is visualised in Fig. 6. Each sample was photographed in the experimental setup previously described. Raw images (.CR2) and the raw2hdr command were used to create the HDR images (Step 2 in Fig. 6). The command typically used in the literature, hdrgen, requires a response curve that could not reliably be generated in the experimental scene, characterised by a limited luminous range and by the presence of electric lighting only. After the creation of the HDR image, the luminance values were saved in matrix form using the Radiance pvalue command (Step 3 in Fig. 6). From this matrix, we extracted and compared the rows corresponding to the image pixels located in the middle of the halo area and intersecting its centre (Step 4 in Fig. 6). Using a python script, a simple moving average function (on a 50 pixels window) was applied to smooth the luminance values over this central area, in order to remove the noise caused by dust particles deposited on the samples. The halo size and intensity could then be quantified and compared across the tested samples by plotting them in logarithmic scale (Step 5 in Fig. 6). The measurements is reported in luminance (candela/m2), but it is not translated in haze percentage.
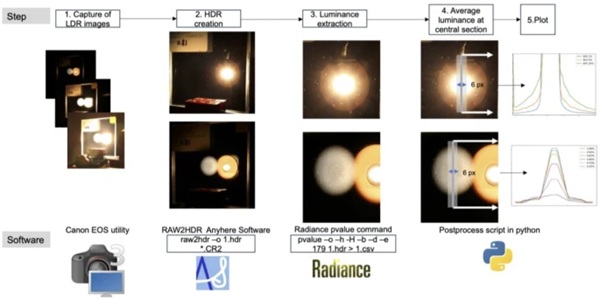
Once the HDR images were produced and luminance values per pixel computed, the ranking of the samples was performed by evaluating the luminous intensity of the halo. Alternative the geometrical distribution and dispersion of the halo could be also used a proxy, but this was not explored in this work.
3 Results
The nine tested samples were compared to each other based on the luminance values extracted from each HDR image and used to characterise the halo typical of haze effects. The results presented here show different setup configurations assessed to understand which one produces a robust and consistent ranking of the samples, when comparing it to the reference values measured by a haze meter.
Figure 7 shows the results for three different light intensity settings (configurations C, B, and A from Table 3), with a fixed distance of 50 cm (‘far’ configuration) between the camera and the sample. It is clear that with the minimum light intensity setting (340 W/m2) the ranking of the samples is not robust and precise enough, as the peak luminance values for the couples of samples 0.92–1.06% and 0.53–0.60% cannot be clearly differentiated. Using medium and maximum light intensities (respectively 2162 and 4325 W/m2) the difference in peak luminance becomes noticeable and it is easier to associate each sample to its own characteristic value. It should be noticed, however, that the ranking is not consistent with the reference values.
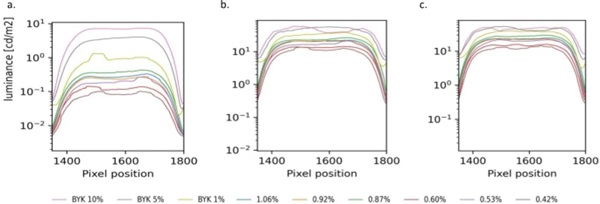
Figure 8 shows the results for three different distances between the camera and the samples (condition E, D and A from Table 3), while the related luminance images are shown in false colour in Fig. 9. The light intensity was kept to the maximum settings in all three cases. The difference in peak luminance values is better distinguishable for the ‘close’ setting (a), in which the camera was placed close to the sample. The sample ranking based on the peak intensity became also more closely aligned with the reference ranking, except for sample 0.92% which resulted in lower values than expected.
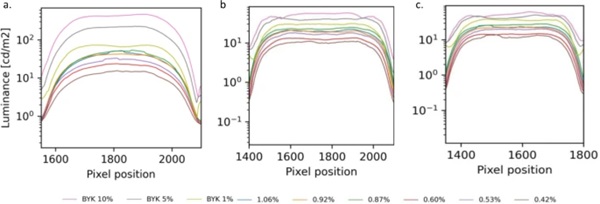
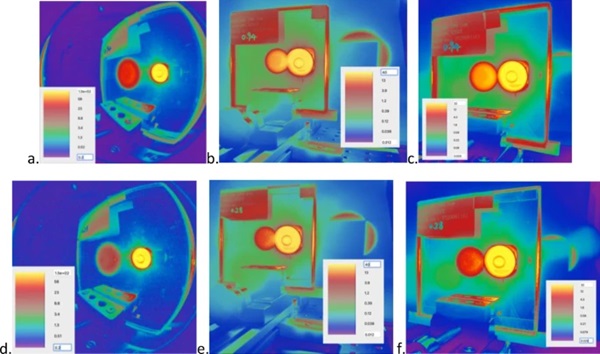
Lastly, the peak luminance values extracted for each sample and configuration are shown in Fig. 10, which summarises the results of the entire experiment. It can be noticed the ranking from the closest position of the camera shows the best agreement with the haze meter ranking. However, the sample with the haze level of 0.87% and 0.53% shows some inconsistency with respect to the ranking performed by the haze meter. This could be due to the lack of resolution of the method with level of haze below two decimals. It can also be noticed from Fig. 3 that the sample 4 seems to exhibit larger haze than sample 5, when the light is incident laterally on the sample. Nevertheless, while not being able to accurately rank samples with a smaller absolute difference than 0.10%, haze differences as small as 0.11% were accurately ranked.
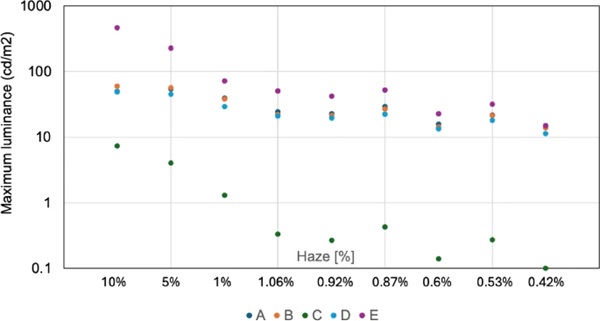
4 Discussion
The core aim of this work was to devise a haze characterisation technique that is low-cost, robust, repeatable, and practical for in-situ assessments. The luminance-based characterisation technique devised for this work was found to effectively identify haze higher than 1%. The method was successful in ranking the samples with haze level larger than 1% and relatively differences between 0.1 and 1 in order of magnitude. Haze measurements below this threshold are affected by the uncertainty that is characteristic of this technique (± 10%) and cannot be reliably distinguished, in particular, when the order of magnitude below 0.1. Our assumption is that this threshold can be taken as representative of the human visual sensitivity as well, i.e. it represents the threshold under which any haze effect would not be noticeable by an average observer. However, this assumption will require further testing with human participants to be proved.
The experiments also allowed us to identify the parameters that can be used to characterise halos and to consequently quantify the severity of haze effects. The parameters that could be clearly identified from HDR images were the halo peak luminance and the halo size. The possibility to correlate haze effects with a new metric that combines these two parameters will be investigated in future work.
The intensity of the light and the distance of the camera have an impact on the accuracy of the results. Closer camera positions and higher light intensity were the most successful in correctly ranking the hazy samples.
5 Conclusion
This paper aims to propose and preliminarily evaluate the potential of luminance-based measurements of the halo induced by light shining on glazing as a proxy for the severity of haze and the related visual nuisance perceived by the observer. A controlled laboratory test was designed to compare the haze severity ranking of different samples, measured both through a standard haze meter and the newly proposed method. The impact of light intensity and light source distance was also assessed by varying these parameters in the test conditions.
The proposed method successfully ranked samples differing in haze intensity by more than 0.1 orders of magnitude, while below this threshold, a noticeable loss in accuracy was observed. Specifically, the method appears to accurately rank samples with haze levels greater than 1%.
There are several aspects that require further investigation to devise the proposed in-situ methodology. This includes the evaluation of potential correlations between halo size and level of haze, the method with samples with a larger variance of haze defect and with more complex glazing build-ups. The samples evaluated in this study were all single glazing, while double or triple glazing configurations should also be considered. Secondly, the impact of the surrounding lighting environment should also be considered when transferring the method to in-situ non-controlled settings. In this sense, the combination of the luminous intensity with the geometrical distribution of the halo instead of the solely use of the luminous intensity could yield more accurate and precise results, especially when comparing haze under different luminous conditions.
Finally, it is still unclear what is the level of resolution required to effectively capture the visual nuisance perceived by the human eye in hazy glazing. This will depend on the surrounding lighting conditions and view content. If the threshold of human sensitivity to haze is established, we will then be able to adequately evaluate the effectiveness of existing and new proposed methods in describing the human experience with haze in glass facades.