Article Information
- Digital Object Identifier (DOI): 10.47982/cgc.9. 605
- Published by Challenging Glass, on behalf of the author(s), at Stichting OpenAccess.
- Published as part of the peer-reviewed Challenging Glass Conference Proceedings, Volume 9, June 2024, 10.47982/cgc.9
- Editors: Christian Louter, Freek Bos & Jan Belis
- This work is licensed under a Creative Commons Attribution 4.0 International (CC BY 4.0) license.
- Copyright © 2024 with the author(s)
Authors:
- Paolo Matricardi - Delft University of Technology, Faculty of Civil Engineering and Geosciences
- Marc Ottele - Delft University of Technology, Faculty of Civil Engineering and Geosciences
- Christian Louter - Delft University of Technology, Faculty of Civil Engineering and Geosciences
- Alessandra Luna Navarro - Delft University of Technology, Faculty of Architecture and the Built Environment
Abstract
This paper focuses on the environmental impact of passive smart windows, novel high-performance glazing technologies that can change their solar transmittance to control the amount of solar gain, thus reducing cooling energy demand. Despite the large influence of building envelope technologies on the overall embodied carbon in buildings, the environmental impact of passive smart windows has been insufficiently addressed, with a dearth of numerical data on various impact categories beyond energy consumption and Global Warming Potential (GWP). While current literature focuses on the advantages of these technologies in terms of operational energy savings, other critical environmental considerations are currently missing. The objective of this paper is to bridge this gap by introducing a novel framework for assessing the broader environmental impact of passive smart windows through a multi-category Life Cycle Assessment (LCA) method. The framework is based on a systematic literature review of current state-of-the-art approaches and Interviews with key stakeholders in the dynamic glazing value chain. Interview results are presented, and then the framework is demonstrated through a case study of thermochromic technologies for an office building in the Netherlands. Preliminary results show the critical areas where improvements in the methods or the performance of the technology are required for the achievement of holistically sustainable high-performing glazing. The service life of the Auto-Responsive (AR) layer and its integration into the Passive Smart Window (PSW) emerge as the most significant factors for reducing the environmental impact of passive smart windows. It's noted that potential benefits in operational energy might not fully offset embodied impact factors.
1.Introduction
Climate change is stressing the importance of reducing the environmental impact of the building and construction sectors, which are responsible for up to 38% of global CO2 emissions (Hamilton & Rapf, 2021). Considering the overall energy consumption of buildings, operational energy is usually, by far, the highest contributor, estimated to be around 80 and 90% of the total (Ramesh et al., 2010). These emissions are strongly influenced by the building envelope, which is the part of the building responsible for mediating between the indoor and the outdoor environment. In particular, the transparent fraction of the facade is responsible for approximately 30% of the overall energy demand of the building (Hee et al., 2015), especially in summer where undesired solar gains can increase cooling demand. The control of solar gains is becoming increasingly important also because of climate change and the related increase in temperature. However, maintaining transparency in the glazed envelope is crucial for providing natural light, outdoor views, and enhancing occupants' well-being (Ko et al., 2020; Woo et al., 2021). Furthermore, transparency plays a vital role in architectural expression (Ko et al., 2020; Woo et al., 2021).
As potential solutions, novel dynamic technologies have been increasingly introduced in the market to enable transparency and simultaneously dynamically control solar gains. These technologies can be either automated or actively controlled, or passive smart window (PSW). These latter systems rely on the intrinsic capability of materials to respond to changes in environmental conditions with property changes (Wang & Narayan, 2021). For instance, by changing the solar or visual transparency, or their colour. Examples of these technologies are thermochromic (TC), thermotropic (TT) or photochromic (PC) glazing (Michael et al., 2023). Passive smart glazing technologies offer the advantage of directly responding to environmental changes without requiring active control, thereby reducing the need for maintenance and additional components.
Most of studies about passive smart windows refer to small-scale production, more at the level of laboratory experiments and prototypes, and are not yet ready for the market (Ke et al., 2019). So far, the researchers have focused mainly on the improvement of the operational performances of dynamic behaviour (Cuce & Riffat, 2015), but few real-scale applications are known due to ease of production. In addition, it is unclear the overall environmental impact of these components, which could undermine their effectiveness in decreasing the environmental impact of the building sector. Only one study was found to estimate the effect of passive smart glazings (Sirvent et al., 2022) on the energy consumption and Global Warming Potential (GWP). However, no information was found about other environmental impact categories.
Passive smart glazing can be made of VO2 based nanocrystals (vanadium dioxide, usually doped with tungsten, titanium and other substances), liquid crystals, ionic liquids, perovskites and hydrogels. Photochromics instead are usually compounds based on noble metals, transition metal oxides (WO3, TiO2, MoO3, V2O5, Nb2O5) silica or organic compounds (fulgides, spiropyrans, pirooxazines, naphthopyrans, and azobenzenes). Currently, it remains unclear whether these materials pose excessive environmental impacts, whether during their production, disposal, or hindering disassembly and recycling of insulating glazing units. This ambiguity is a common challenge with new high-performance facade technologies, which often incorporate multiple materials that are challenging to disassemble, thereby limiting their potential for reuse or recycling (Hartwell et al., 2021). The objectives of this paper are to: (i) develop an appropriate framework for conducting a Life Cycle Assessment (LCA) to evaluate various Environmental Impact Categories across different phases of the product life cycle; and (ii) offer an initial quantitative assessment of the overall environmental impact of passive responsive glazing.
To achieve the first objective, a literature review and interviews are conducted to inform the design of the evaluation framework. This involves synthesizing existing research and gathering insights from stakeholders to establish a comprehensive approach to assess environmental impacts across the life cycle of passive responsive glazing.
For the second objective, the LCA methodology is applied to a case study of an office building located in the Netherlands. This entails analysing the environmental footprint of passive glazing within the context of the building's overall environmental impact, providing valuable insights into its sustainability implications.
Upon reviewing existing literature on the LCA of windows, it became apparent that comprehensive details necessary for framing the methodology were lacking. Particularly, crucial information regarding the End-of-Life (EoL) stage of glazing was absent, hindering accurate modelling of its impact within the window life cycle. Additionally, the integration of passive layers into glazing build-ups and their treatment at the EoL stage, given current infrastructure constraints and related environmental implications, remained unclear.
To address these knowledge gaps, interviews were conducted with experts from the industrial sector to validate existing assumptions and enrich the framework with additional insights. Section 2 outlines the methodology used to develop the assessment framework, including the approach for conducting interviews and performing the LCA. Section 2.2 presents the chosen case study for demonstration purposes.
In Section 3.1, results from the expert interview sessions and related assumptions drawn from this qualitative data collection are reported. Section 3.2 details the outcomes of building performance simulations, while Section 3.3 presents the findings regarding embodied impact. Section 3.4 provides the overall results of the LCA.
Finally, conclusions are drawn in Section 4, summarizing the key findings and implications of the study.
2. Methodology
2.1. Interviews
Seven interviews were conducted, with experts spanning various disciplines within the flat glass and facade industry. The questions asked were designed to address the knowledge gaps identified in the literature review and to gain insights into current industry practices. The interviews focused on three main themes: the existing flat glass recovery system and its characteristics; the production of PSW, the integration of the AR layer, potential failure causes, and the glazing EoL scenarios; and the application of LCA in evaluating facade products, including the selection of Life Cycle Impact Assessment (LCIA) methods, data sources, and techniques for uncertainty calculation. Comparisons between responses from different interviewees were made where possible to enhance reliability. Figure 1 illustrates the interviewees and the topics they contributed to, names and companies are not reported to ensure privacy.
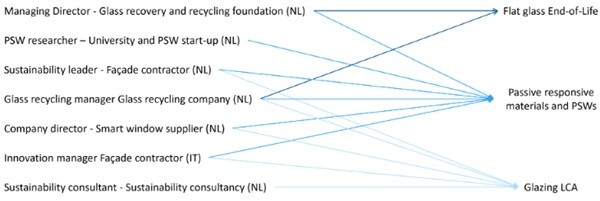
2.2. Case study
The study compares two window systems, a PSW and a DWS (Dynamic Window System) The first is aPSW is modelled after Suntuitive Low-Iron with 3 low-e coatings (Pleotint, 2019), with a thermochromic material embedded in the interlayer, assumed to be VO2 powder. The DWS consists of two components, a static window (StW) and a manual indoor blind, thus avoiding active energy consumption. Therefore, both technologies consist of a static glazing and a dynamic component, the layer for PSW and the blind for DWS. The StW was calculated as well and used as benchmarking technology. The windows were assumed to be manufactured in the US, where Pleotint is located, used in a building in the Netherlands and disposed of in the same region.
The Embodied impact was calculated through an LCA, while the Operational impact was estimated through an energy simulation. These were finally summed using a baseline attribution approach: StW results were subtracted from the results of PSW and DWS, giving impact variations for both operational and embodied stages. The total impacts of PSW and DWS were then the sum of the respective operational and embodied impact variations.
The operational energy use of the window technologies was determined through energy simulation, using the EnergyPlus23.2.0 software (National Renewable Energy Laboratory (NREL), 2023): 9,6m2 of window were applied in a standard office room of 6x8 m2. All the surfaces are adiabatic except the south-oriented facade, which consists of an opaque wall with a WWR of 44,4%. The insulated brick and concrete wall has a U-value of 0,295 W/m2K, fulfilling the requirements for new buildings according to the Dutch building code Bouwbesluit (2012). Occupancy and equipment load densities were considered, with systems operating only during occupancy hours, thus 5 days a week between 8:00 and 18:00. The occupants are quantified through a density of 0,11 people/m2 and a sedentary metabolic rate of 126 W/person, while the equipment’s load density is 11 W/m2. The lighting has a load density of 6,5 W/m2 and is set to maintain a minimum light intensity of 500 lux at a reference point, positioned in the middle of the room at a height of 0,8 m. The HVAC System is an ideal full air system automatically sized based on the climate of Amsterdam and the thermostat set-points are 19 °C and 26°C. An infiltration of 1,44 V/h is assumed. Coherently with the LCA choices, Amsterdam was chosen as the location for the simulation, taking the corresponding weather file from EnergyPlus repository. Following the conservative approach, the output of the simulation was considered as the total energy demand, calculated as the sum of lighting, heating and cooling. The input for the Operational energy in the LCA was therefore the difference between the energy demand of a technology and the energy demand of StW. All the energy was assumed to be supplied as ”market for electricity, low voltage-NL” from Ecoinvent (Swiss centre for life cycle inventories, 2022).
Figure 2 illustrates the build-ups of the three windows, all consisting of a Double Glazing Unit (DGU) with laminated external pane and low-e coating on the 5th face and modelled with data provided by version 81 of the International Glass DataBase (2021), the latest including the data for Suntuitive.
- The thermochromic glazing of PSW (Figure 2b) is modelled using the spectral data of different tinting states of the glazing.
- The optical properties of the blind of DWS (Figure 2c) were taken from the GreenScreen-Eco (HunterDouglas) and its activation is modelled on the behavioural model by Haldi and Robinson (2009).
The system boundary in the LCA was defined through a cradle-to-gate approach, using a “cut-off system model”, thus neglecting the benefits of recycling or side products. The LCA calculation of the embodied impact made use of the OpenLCA2.1 software, while the data were taken mainly from Ecoinvent 9.3.1 database (2022). The LCIA method Environmental Footprint 3.1 was used, with normalization (Andreasi Bassi et al., 2023) and weighting factors (Sala & Cerutti, 2018) leading to a final Single Score, as developed by the JRC of the European Commission (2021).
Only the dynamic components of the technologies and the related processes are considered as foreground: (i) for the PSW, this includes the production of 1,14 g of VO2 per m2 of glazing, based on works by Sirvent (2022) and Weber (2018), the additional interlayer material (EVA) and the EoL of VO2; (ii) For DWS, the specific processes consisted in the production, construction and EoL of the blinds, derived from an EPD (Epddenmark, 2023) and assumptions on transport distance.
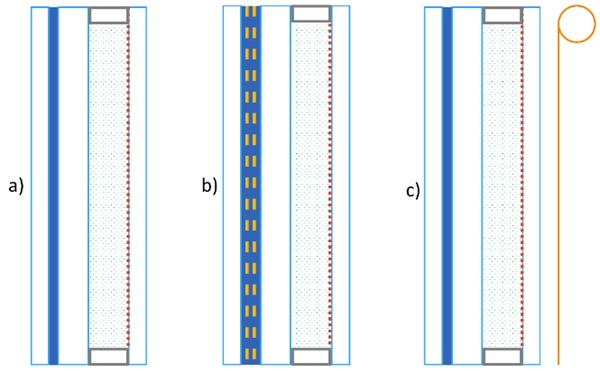
Table 1: Environmental impact categories from EF3.1 and respective acronyms used in this study.
Concerning the life stages, most of the modules of the Use stage were neglected, as were installation and removal of windows and blind on site. Waste treatment and disposal modules (C3 and C4) were addressed together in the EoL stage. Based on the interviews and literature review, the chosen lifespan of the LCA was 45 years.
The Replacement (B4) was modelled as repetition of the other embodied stages (Production, Construction and EoL) multiplied by the replacement rate. Therefore, the replacement is computed as the ratio between the service life of the product divided by the lifespan considered. An important distinction was made between PSW and DWS: since the AR layer is embedded in the PSW glazing, the whole PSW must be replaced when the AR layer fails, so the product has one single replacement rate. DWS instead consists of two separate components, the StW and the blind, which can be replaced separately and thus have their own replacement stage with respective replacement rates; this is reflected in the LCA modelling (Figure 3).A baseline scenario was drawn and analyzed in detail, while other scenarios concerning the service life of both the VO2 and the blind and the EoL of the glazing. The baseline service life of the PSW was assumed of 20 years, thus shorter than the static window’s (30 years) but longer than the blind’s (15 years).
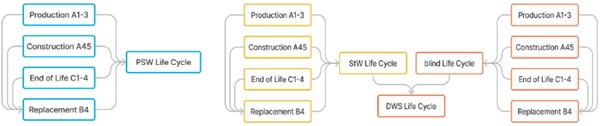
The glazing recycling impact was estimated based on the data obtained from the interviews and the annual report of Vlakglas Recycling Nederland (VRN) (Wittekoek, 2023), while the landfill was based on Ecoinvent data. Concerning the impact modelling of VO2, strongly conservative assumptions were made, due to a lack of sources: in both scenarios, the whole mass of VO2 was assumed to impact on the environment, avoiding any residue in the interlayer material, and modelled as Vanadium (not vanadium dioxide). For the landfill scenario, the mass was assumed to leak from the interlayer to the soil; for the recycling scenario, it was assumed to be dispersed in the air due to incineration of the interlayer.
3. Results and discussion
3.1. Interviews
Flat glass recycling. Through interviews, it became evident that flat glass recycling encompasses two primary streams: Post-Industry Recycling (PIR) and Post-Consumer Recycling (PCR). PIR addresses waste generated during flat glass manufacturing, while PCR manages EoL flat glass from buildings. Some countries, such as France and the Netherlands, have implemented PCR recovery systems to reintegrate flat glass into the value chain. The Netherlands, in particular, leads in flat glass recycling, with approximately 80% of PCR collected. The flat glass recycling process involves crushing glass into cullet, which is then sorted to remove impurities, especially through optical sorting, which is crucial for removing contaminants using sensors; however, this process may also eliminate clean glass fragments. In the Netherlands, 91.3% of collected flat glass waste is recycled and used for glass envelopes and insulation. Yet, the high contaminants content of PCR glass often hinders the reintroduction of its cullet in flat glass production. Glass coatings are generally not problematic during recycling, as they evaporate at melting temperatures but concerns arise regarding potential reactions within the furnace, especially in cold spots, which could damage furnace materials.
Production and integration of passive materials in windows. Passive materials and production methods vary depending on their application, with photochromic and thermochromic technologies being prominent. Photochromic windows predominantly use inorganic compounds like metal oxides, while thermochromic ones often rely on organic compounds like liquid crystals. Production involves hydro-thermal reactions followed by embedding particles in polymeric layers or coatings, with energy consumption being a significant factor. In current static window practices, the demand for glazing types varies regionally and according to building size and purpose, with a prevalence of laminated DGU; moreover, integrated shading solutions are rare and indoor blinds represent the most commonly used device. For PSWs, three main integration options exist, including coatings, interlayer films, and adhesive films, each with different applications and protection levels. Lifespan considerations vary across markets, with Italian facades lasting around 50 years and UK ones often replaced within 20-30 years. Shading systems can last up to 60 years with proper maintenance, while the service life of PSW ranges from 5 to 10 years for adhesive films and up to 10 years for interlayer coatings, due to the different failure mechanisms. EoL scenarios for AR layers involve challenges in recycling due to integration methods. Adhesive AR layers can be removed before glass recycling and AR interlayers can be separated when the glass is fragmented, but AR coatings pose difficulties due to lack of established removal techniques. Specifically, higher attention must be paid to liquid crystal compound, which pose additional challenges due to legislation and compatibility issues with recycling processes. Overall, understanding the production methods, integration options, service life, and EoL considerations of the used AR material is crucial for reliably estimating the impact of its integration in glazing.
Assumptions for LCA. The workflow adopted by the façade contractor and the sustainability consultant were different due to their divergent roles in the industry and, consequently, their different aims in the LCA. These also determined a different coverage of the product life cycle, with the sustainability consultant considering the entire life cycle, while the façade contractor focuses on assembly and construction, and different LCIA methods. Transportation assumptions vary, with the sustainability consultancy suggesting to refer to the PEF framework for unknown locations; however, their impact is considered quite low. Data gaps and uncertainties pose challenges and should be addressed by relying on manufacturers' data and proxy assumptions and modelling new materials based on similar ones.
Both interviewees mentioned that it is challenging to integrate operative and embodied energy consideration in the LCA and the results should be presented separately for clarity.
Addressing data gaps transparently is crucial for LCA's credibility. Lifespan considerations are significant, with façade contractors assuming the facade lifespan matches the most durable components. Replacement scenarios are not clear, a façade contractor suggested to separately define for each component. Alternatively, the full facade element can be replaced after failure of the first component. EoL modelling faces uncertainties: multiple scenario analyses should be performed, starting with a conservative baseline scenario as suggested by a sustainability consultant. Various waste treatment options can be combined for components, with impacts calculated accordingly. End of Life data can be obtained from the Nationale Milieudatabase, including benefits from incineration, reuse, or recycling, but with assumptions due to contractor limitations.
3.2. Operational energy
The calculated annual energy demands for lighting, heating, and cooling are shown in Figure 4. Both PSW and DWS increased the lighting energy demand as expected since they reduced the incomingsolar radiation. The significant disparity between the technologies is attributed to their functioning: manual blinds (+36%) are operated by users and are either active or inactive, thus screening rejecting more energy than necessary, while the PSW (+5%) can assume several intermediate states, with a finer control of the solar gains. Cooling demand decreased with PSW (-31%), while manual DWS showed negligible savings, probably due to the occupant behavioural model applied which is also driven by satisfaction with daylight. The heating demand increased in both cases due, but this was expected, since the activation of the blind and the reaction of PSW are not optimal. Overall, PSW slightly reduced total energy demand, while DWS increased it, primarily due to its impact on heating and lighting. Cooling and heating demands were very similar to lighting energy demand due to the high performances of the building envelope and the conservative HVAC set-point range, which reduced the demand for HVAC systems.
3.3. Embodied energy and environmental impact
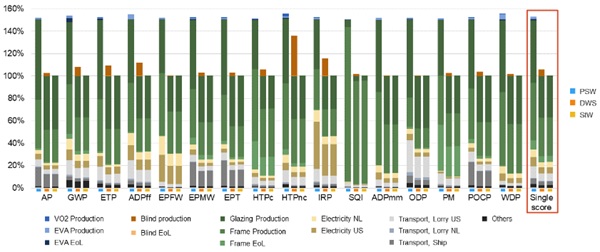
The Embodied impact of the product systems was calculated through an LCA. When analyzing the contribution of different impact categories to the weighted Single Score of each product system, it was found that five impact categories - GWP, ADPff, PM, ADPmm, and AP - accounted for nearly 75% of the impact for both PSW and DWS. Figure 4 shows the process contribution to the embodied impact of PSW, DWS and StW for the baseline scenario, normalized on StW.
Observing the total results, PSW consistently has higher embodied impact than StW by around 50%; DWS has higher values than StW as well but less consistently and less remarkably across all the categories. The reason for these differences is explained by the contribution analysis. As Figure 4 shows, most of the embodied impact of both PSW and DWS derives from processes that are shared and are related to the production of the static window StW. These processes entail components production and EoL, electricity consumption for frame assembly and disassembly and transportation. The processes that are specific to each product system are responsible for negligible impacts. The processes required to produce the PSW only represent an increase of 3% on the Single Score. The production of blinds in the DWS is responsible for an increase of the 6% on the Single Score, while for larger increases in specific indicators (12% for the ADPff, 8% for the GWP, 36% for the HTPnc and 16% for the IRP.
The differences in service life have a significant impact on the LCA. The PSW shows a significantly larger contribution of the common processes, due to the lower service life of the PSW: during the LCA lifespan, the PSW is fully replaced twice, conversely to the StW that is only replaced once. The difference in expected life of the PSW is responsible for the 50% increase on each impact category. This highlights the relevance of the service life of the PSW in comparison to static windows.
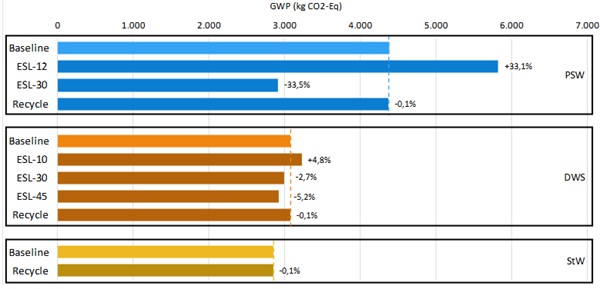
The importance of the service life was confirmed by the analysis of the scenarios, reported in Figure 5. For PSW, the service life variation determines the replacement rate of the whole product, thus causing high variation in its impact, increasing it or decreasing it by around 33%, while for the replacement of the DWS’ blind the impact variation is lower. This discrepancy is easily explained by the different build-up of the two technologies: the blind can be replaced independently from the window, while for PSW the integration of the AR layer within the glazing determines the replacement of the whole product. This distinction suggests the separability of components of the window system can reduce its impact; this is achieved for PSW as well when the AR material is applied on a detachable film, despite its shorter service life.
The recycling scenario instead, evaluated for PSW, DWS and StW, gave negligible variations in GWP as well as in all other categories, with a maximum decrease around -0,6% (ODP), thus confirming the negligible impact of the EoL of the glazing.
3.4. Total results
In the final step, the total impacts for PSW, DWS and StW were calculated by summing operational and embodied impacts. Subsequently, for PSW and DWS, the variations in impact relative to StW were determined, thus adopting a baseline attributional approach. The standard deviation of operational impacts was assumed to be 15% of the result, based on the study by Li et al.(2023), while a Monte Carlo simulation was used to determine the values and standard deviation of the embodied impacts, according to the procedure described by Owsianiak et al. (2018). As anticipated, DWS consistently increased the environmental impact of StW since increased the operational energy demand. Conversely, PSW exhibited a lower operational impact than StW, resulting in a decrease in the total impact.
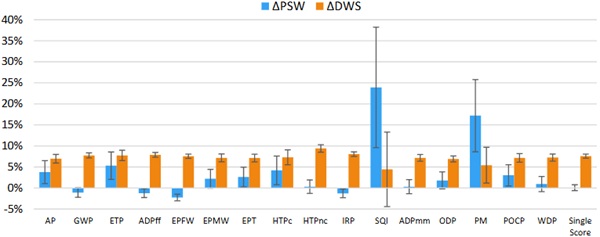
In Figure 6, the total impact variations of PSW and DWS in relation to StW are illustrated, showcasing their standard deviations. ΔDWS consistently showed a positive value, thus an impact increase, while ΔPSW exhibited more varied outcomes. In most categories, including the most significant ones and the Single Score, ΔPSW was lower than ΔDWS, indicating a lower total impact with the use of Passive Smart Windows due to energy demand savings during the operational stage.
Notably, while the operational impact was expected to outweigh the embodied impact for PSW, leading to a negative ΔPSW, the findings revealed a positive value on several categories. This suggests that the increase in embodied impact is not adequately compensated by operational energy savings. Such unexpected results may stem from over-conservative assumptions; among these, the choice of a high performance building for the energy model, may have reduced the absolute values of the energy demands and thus the difference between the demand of PSW and StW, contributing to this discrepancy.
4. Conclusions
This study aimed to develop a framework for assessing the environmental impact of Passive Smart Windows (PSW) using the Life Cycle Assessment (LCA) approach, considering both operational and embodied impact differences. The framework, initially defined through literature research and expert interviews, was applied to a case study using the LCIA method Environmental Footprint 3.1 to compare a thermochromic PSW to a Dynamic Window System (DWS), consisting of a Static Window and an indoor blind. The total impact difference between the technologies and a benchmarking static window (StW) was calculated.
The main impact categories included Global Warming Potential (GWP), Abiotic Depletion Potential from fossil fuels (ADPff), Particulate Matter (PM), Abiotic Depletion Potential from minerals (ADPmm), and Acidification Potential (AP), contributing to nearly 75% of the Single Score. PSW generally exhibited a lower total impact than DWS across most categories, but it showed a higher total impact compared to StW, primarily due to operational impacts being less significant than expected.
Factors such as building performance characteristics, climate conditions, and a wide temperature set-point range increased lighting demand relative to heating and cooling, altering expected energy savings. Simulation outputs were deemed excessively conservative, diminishing the significance of operational impacts. The study highlighted challenges in decoupling product systems from the application context due to limited data availability and LCA assumptions.
Embodied impacts were significant, with production stages contributing the most to the overall impact. PSW's Expected Service Life was identified as the most influential factor of the embodied impact: the integration of passive materials in the glazing dictates whole glazing replacement since it is fully integrated. For the DWS, the replacement of the blind doesn’t affect the window, suggesting that a similar solution for PSW would significantly decrease its embodied impact. The increase of energy demand related to the use of manually operated DWS instead of STW showed that occupant behavior can make these systems inefficient. Further research should explore the impact of automated control of blinds in comparison to StW and PSW.
Obstacles in LCA modelling included limited information and data on the impact of the EoL of the glazing and passive materials, especially for their EoL, leading to strong assumptions and increased uncertainty. The Single Score simplified the analysis, highlighting relevant impact categories, though biases in weighting sets required consideration.
The assessment focused solely on the energetic performance of the operational phase, disregarding other important aspects such as glare control and user activation. To fully understand PSW's impact, a more comprehensive evaluation should consider users’ comfort in terms of glare and interaction with the facade, and long-term adaptability to changing weather conditions.
References
Andreasi Bassi, S., Biganzoli, F., Ferrara, N., Amadei, A., Valente, A., Sala, S., & Ardente, F. (2023). Updated characterisation and normalisation factors for the Environmental Footprint 3.1 method. In Publications Office of the European Union (Vol. JRC130796). https://doi.org/10.2760/798894
Bouwbesluit Online (2017), 416 Ministerie van binnenlandse zaken en koninkrijksrelaties (2012).
Cuce, E., & Riffat, S. B. (2015). A state-of-the-art review on innovative glazing technologies. Renewable and Sustainable Energy Reviews, 41, 695–714. https://doi.org/10.1016/j.rser.2014.08.084
Epddenmark. (2023). EPD of Rollerblind system by Fisher. https://www.epddanmark.dk/media/qk5h4fgc/md-23013-en.pdf
European Commission. (2021). Annexes 1 and 2 to COMMISSION RECOMMENDATION on the use of the Environmental Footprint methods to measure and communicate the life cycle environmental performance of products and organisations. https://environment.ec.europa.eu/document/download/680503dc-5a19-4f6a-bb92-84d9bfc8f312_en?filename=Annexes%201%20to%202.pdf
Haldi, F., & Robinson, D. (2009). Interactions with window openings by office occupants. Building and Environment, 44(12), 2378–2395. https://doi.org/10.1016/j.buildenv.2009.03.025
Hamilton, I., & Rapf, O. (2021). 2020 Global Status Report for Buildings and Construction: Towards a zero-emissions, efficient and resilient buildings and construction sector: Excutive Summary. In Global Alliance for Buildings and Construction. https://wedocs.unep.org/20.500.11822/34572
Hee, W. J., Alghoul, M. A., Bakhtyar, B., Elayeb, O., Shameri, M. A., Alrubaih, M. S., & Sopian, K. (2015). The role of window glazing on daylighting and energy saving in buildings. Renewable and Sustainable Energy Reviews, 42, 323–343. https://doi.org/10.1016/j.rser.2014.09.020
HunterDouglas. (n.d.). RB 500 Roller Shades Specification Catalog. Retrieved February 16, 2024, from www.hunterdouglascontract.com/windowcoverings
Ke, Y., Chen, J., Lin, G., Wang, S., Zhou, Y., Yin, J., Lee, P. S., & Long, Y. (2019). Smart Windows: Electro-, Thermo-, Mechano-, Photochromics, and Beyond. Advanced Energy Materials, 9(39), 1902066. https://doi.org/10.1002/AENM.201902066
Li, J., Lützkendorf, T., Balouktsi, M., Bi, X., Alaux, N., Potrč Obrecht, T., Passer, A., Han, C., & Yang, W. (2023). Identifying uncertainties in the whole life carbon assessment of buildings: Sources, types, and potential actions. Building and Environment, 244, 110779. https://doi.org/10.1016/J.BUILDENV.2023.110779
Michael, M., Favoino, F., Jin, Q., Luna-Navarro, A., & Overend, M. (2023). A Systematic Review and Classification of Glazing Technologies for Building Façades. In Energies (Vol. 16, Issue 14). https://doi.org/10.3390/en16145357
National Renewable Energy Laboratory (NREL). (2023). EnergyPlus. https://energyplus.net
Owsianiak, M., Bjørn, A., Bugge, H. B., Carvalho, S. M., Jebahar, L., Rasmussen, J., White, C. M., & Olsen, S. I. (2018). Illustrative Case Study: Life Cycle Assessment of Four Window Alternatives. In Life Cycle Assessment (pp. 1059–1146). Springer International Publishing. https://doi.org/10.1007/978-3- 319-56475-3_39
Pleotint. (2019). Suntuitive dynamic glass - Technical infromation. https://suntuitiveglass.com/wp-content/uploads/2019/11/Tech-broch.25112019_PL_New.pdf
Ramesh, T., Prakash, R., & Shukla, K. K. (2010). Life cycle energy analysis of buildings: An overview. Energy and Buildings, 42(10), 1592–1600. https://doi.org/10.1016/J.ENBUILD.2010.05.007
Sala, S., & Cerutti, A. K. (2018). Development of a weighting approach for the Environmental Footprint. https://doi.org/10.2760/446145
Sirvent, P., Tanguy, A., Pérez, G., Charriere, R., & Faucheu, J. (2022). Environmental Benefits of Thermochromic VO2 Windows: Life Cycle Assessment from Laboratory Scale to Industrial Scale. Advanced Engineering Materials, 24(4), 2101547. https://doi.org/10.1002/ADEM.202101547
Swiss centre for life cycle inventories. (2022, December). Ecoinvent Database v3.9.1. http://www.ecoinvent.org/database/
Wang, X., & Narayan, S. (2021). Thermochromic Materials for Smart Windows: A State-of-Art Review. Frontiers in Energy Research, 9. https://doi.org/10.3389/fenrg.2021.800382
Weber, S., Peters, J. F., Baumann, M., & Weil, M. (2018). Life Cycle Assessment of a Vanadium Redox Flow Battery. Environmental Science & Technology, 52(18), 10864–10873. https://doi.org/10.1021/acs.est.8b02073
Wittekoek, M. (2023). Jaarverslag 2022 - Vlakglas Recycling Nederlands. https://www.vlakglasrecycling.nl/uploads/jaarverslagen/Jaarverslag%202022_VRN_4.pdf